The World of Bacteria
Bacteria are microscopic, single-celled organisms that grow in various environments. They can live in the soil, oceans, and even inside the human gut.
The relationship between humans and bacteria is complex. Sometimes, bacteria help us by transforming milk into yogurt or aiding in digestion. In other cases, bacteria are harmful and cause diseases like pneumonia from methicillin-resistant Staphylococcus aureus.
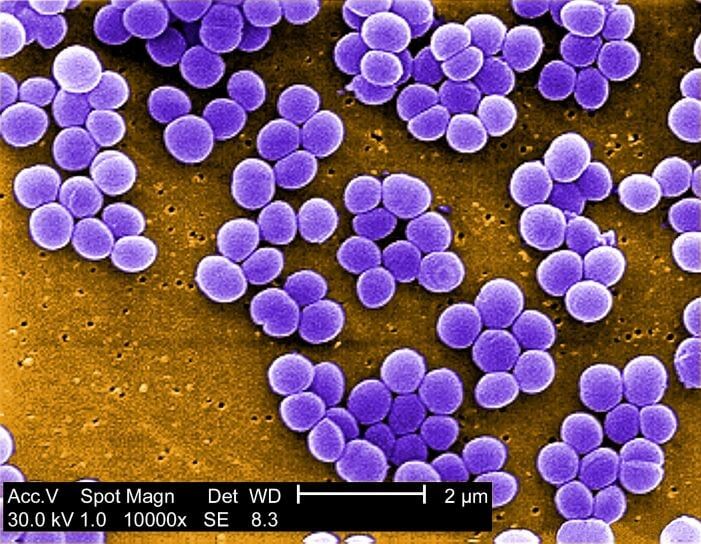
Bacteria are classified as prokaryotes, meaning they are single-celled organisms that contain DNA freely floating in a twisted, thread-like mass called a nucleoid. Ribosomes are the global units in bacterial cells where proteins are assembled from amino acids using information encoded in ribosomal RNA.
Bacterial cells are generally surrounded by two protective layers: an outer cell wall and an inner cell membrane. Some bacteria, like Mycoplasma, lack a cell wall. Some bacteria may even have a third outer protective layer called a capsule.
Flagella-like extensions typically cover long bacterial surfaces, helping them move (the longer ones are called flagella, and the shorter ones are called pili) and attach to a host.
Bacteria can be both beneficial and harmful to human health. Commensal or “friendly” bacteria share space and resources in our bodies and tend to help us. There are about ten times as many microbial cells in our bodies as human cells. According to microbiologist David A. Relman’s article in Nature magazine in 2012, the highest number of microbial species is found in the intestines.
The human gut provides a comfortable environment for bacteria, with plenty of nutrients available for them. A 2014 review published in the American Journal of Gastroenterology found that intestinal bacteria, like beneficial strains of E. coli and Streptococcus, help digestion and support the immune system by preventing the colonization of harmful pathogens. Furthermore, the disruption of gut bacteria has been associated with specific disease conditions. For example, a 2003 review published in The Lancet found that patients with Crohn’s disease have an increased immune response against their gut bacteria.
Other bacteria can cause infections. Various bacteria, such as Group A Streptococcus, Clostridium perfringens, E. coli, and S. aureus, can cause rare but severe soft tissue infections known as necrotizing fasciitis (sometimes called the “flesh-eating bacteria”). According to the Centers for Disease Control and Prevention, these infections affect tissues surrounding muscles, nerves, fats, and blood vessels; they are treatable, especially when caught early.
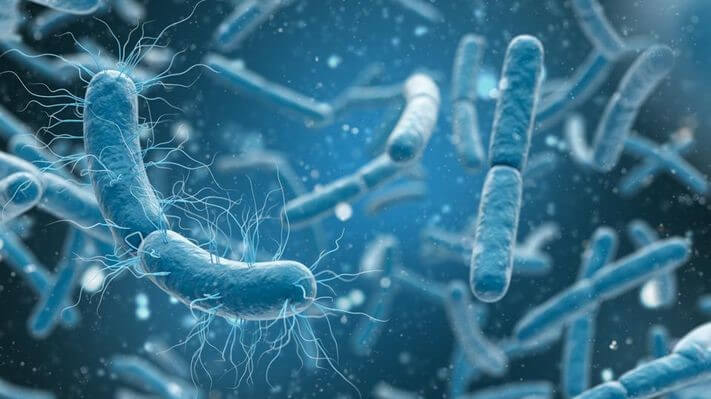
Effects of UVC Rays on Bacteria
Numerous research studies and reports have shown that when biological organisms are exposed to deep UV light in the range of 200 to 300 nm, it is absorbed by DNA, RNA, and proteins.
Absorption by proteins can lead to the rupture of cell walls and the death of the organism. Absorption by DNA or RNA, especially by thymine bases, results in the inactivation of RNA or DNA double helix strands through the formation of thymine dimers. If enough of these dimers are formed in the DNA, the replication process is disrupted, and the cell can no longer multiply.
It is widely accepted that it is not necessary to kill pathogens with UV light, but rather to apply enough UV light to prevent the organism from replicating. The UV dose required to prevent replication is smaller than the dose needed to kill, making the UV process commercially feasible for infection prevention.
Generally, bacteria have developed a number of repair mechanisms to repair UV-induced lesions. These mechanisms include direct reversal of damage by photolyase (photoreactivation), removal of the damaged base by a DNA glycosylase (base excision repair), cutting of the DNA adjacent to the damage by an endonuclease (UV-damaged endonuclease), or complete removal of the oligonucleotide containing the damage (nucleotide excision repair). As a result, the strategy in UV disinfection is to provide a sufficiently high dose to ensure that the nucleic acid is damaged beyond repair.
In disinfection, the Log Reduction Value is measured to determine the inactivation rates. Log reduction is a simple mathematical term used to express the relative number of live microbes eliminated by disinfection.
The UV dose is the amount of UV radiation a bacterial cell is exposed to. UV radiation depends on the intensity of the radiation and the exposure time. A number of biological studies have produced typical UV dose requirements for common target bacteria in disinfection. For example, a dose of 60 mJ/cm² is required to achieve a 3-log reduction (99.9%) for B. subtilis (ATCC 6633).
*Table 1: Log Reduction Value
Log | Factor | Percentage |
1 | 10 | 90% |
2 | 100 | 99% |
3 | 1,000 | 99.9% |
4 | 10,000 | 99.99% |
*Table 2: UV Dose
| Microbial Reduction | Microbial Reduction | Microbial Reduction | Microbial Reduction |
Log Reduction Value | 1 | 2 | 3 | 4 |
MS2(Gram+Bakteri) | 20.0 | 42.0 | 68.0 | 90.0 |
B. Subtilis ATCC 6633 | 20.0 | 39.0 | 60.0 | 81.0 |
E. Coli O157:H7 | 2.0 | 2.0 | 2.5 | 4.0 |
Staphylococcuss aureus | 3.9 | 5.4 | 5.6 | 10.4 |
In 2013, around 140 representatives from the United Nations Environment Programme (UNEP) approved the Minamata Mercury Convention, an international agreement to protect public health and the environment from mercury contamination. This agreement aims to reduce the amount of mercury released into the environment by regulating the production and import/export of mercury-containing products. Once implemented, the use of traditional low-pressure mercury UV lamps will be banned, and the industry will need to adopt new alternative UV emission technologies.
UV-C Light Emitting Diodes are gaining popularity as an alternative technology that overcomes the limitations of traditional mercury-containing UV lamps. For example, their small sizes make it easier to integrate them into sterilization systems, and most importantly, they do not contain mercury, reducing the risk of human and environmental toxicity (01). Shin et al. reported that UVC intensity is not affected by temperature changes and that no heating time is required for maximum intensity output, whereas LP lamps’ output intensity decreases at low temperatures and approximately 5 minutes of heating time is needed (02). Additionally, UVC rays showed significantly higher inactivating activity against Escherichia coli O157: H7, Salmonella enterica serovar typhimurium, and Listeria monocytogenes at the same dosage levels as LP lamps (03). The use of MS2 (Enterobacteria phage), which replaces human enteric virus, was effectively inactivated in batch and continuous-type water disinfection systems containing UV light rays in studies (04).
Airborne transmission of microorganisms plays a significant role in nosocomial infections caused by viruses, bacteria, and fungi (05,06). Exposure to airborne fungi such as Aspergillus, Alternaria, and Cladosporium has been linked to various respiratory diseases (07-09).
In 2003, the Severe Acute Respiratory Syndrome Coronavirus (SARS-CoV) and in 2009, the Swine Flu Virus H1N1 promoted numerous research studies aimed at controlling pathogenic microorganisms, including viruses, bacteria, and fungi, through indoor air disinfection and air purification systems (10-14). To ensure microbial safety of air, several studies have utilized UV rays (15-18). The Centers for Disease Control and Prevention (CDC) recommend UV antiseptic irradiation as an additional procedure to prevent the transmission of tuberculosis-causing bacteria (19).
It is well known that UVC light has a powerful microbial-killing effect and can inactivate a wide range of microorganisms, including viruses, bacteria, protozoa, fungi, yeasts, and algae, through the formation of pyrimidine dimers, which are photo-products of genetic materials (20,21).
Pyrimidine dimerization disrupts DNA replication and transcription, leading to cell death. To date, UV irradiation has mostly been performed with traditional low-pressure mercury UV lamps emitting a peak wavelength of 254 nm (22,23).
In a study, bacterial microorganism inactivation was investigated using a series of UVC light in a room-type air disinfection system. Inactivation rate constants were calculated to analyze the effectiveness of this new UVC irradiation system.
Surviving populations and appropriate curves for E. coli O157: H7, S. typhimurium, L. monocytogenes, and S. aureus are presented in Figure 1. Increasing UVC irradiation dosages resulted in a reduction of bacterial populations. At a dosage of 1.5 mJ/cm² for E. coli O157: H7, a reduction of approximately 2.5 logs was achieved, while for other bacterial pathogens, a reduction of 3 to 4.5 logs was achieved at 4.6 mJ/cm². Only the survival curve of S. typhimurium exhibited an upward concavity among pathogenic bacteria, while a nearly linear decrease was observed for other bacterial pathogens. In bacterial inactivation through UVC irradiation, superior effectiveness was noted in the dose-response value. Lin and Li reported a 2-log reduction in E. coli with traditional UV lamp irradiation at 2.4 mJ/cm² and 80% relative humidity (24). Additionally, S. aureus aerosols in a UVGI system, with an air flow of 30 liters per minute and 87.3% to 90% relative humidity, experienced a 3-log reduction at a dosage of 2,300 mJ/cm² (25).
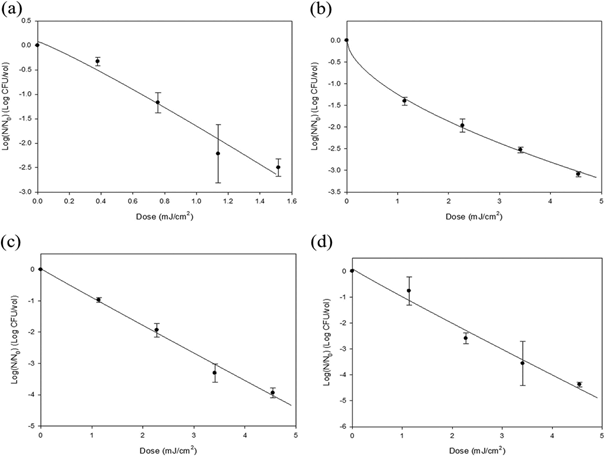
Another study aimed to detect the bacterial density within the air mass of animal shelters. Staphylococcus aureus (DSM 799 strain) was selected because most of the airborne bacteria in animal shelters are gram-positive bacteria. Additionally, the methicillin-resistant variant of S. aureus can be found in large numbers in pigsties. Actinobacillus pleuropneumoniae causes acute, subacute, and chronic respiratory infections in pigs. The A. pleuropneumoniae strain (DSM 13472) was used for the experiments (26, 27).
*Table 3: Reduction in pathogen quantity was determined in an air filter test chamber with and without UVC irradiation.
Pathogen | Test Location | UVC Intensity (μW/cm²) | Relative Humidity (%) | Pathogen Amount | Reduction (%) ± | ||
Culture Suspension | In Front of Filter | Behind Filter | |||||
Staphylococcus aureus | filter | 0 | 15.3 | 7.34 x 108 cfu/ml | 8.31 x 105 cfu/ml | 2.64 x 105 cfu/ml | 67.71 ± 7.41 |
filter, UVC | 1,100 | 3.2c | 6.73 x 108 cfu/ml | 3.43 x 105 cfu/ml | 1.13 x 103 cfu/ml | 99.74 ± 0.57 | |
UVC | 1,190 | 12.7 | 6.42 x 108 cfu/ml | 5.07 x 105 cfu/ml | 4.60 x 101 cfu/ml | 99.99 ± 0.01 | |
Actinobacillus pleuropneumoniae | filter | 0 | 15.3 | 8.73 x 108 cfu/ml | 1.49 x 103 cfu/ml | 7.63 x 102 cfu/ml | 49.43 ± 23.77 |
filter, UVC | 1,220 | 17.4 | 9.40 x 108 cfu/ml | 9.15 x 102 cfu/ml | 0 cfu/ml | 100.0 ± 0.0 | |
UVC | 1,180 | 15.4 | 8.10 x 108 cfu/ml | 5.53 x 103 cfu/ml | 0 cfu/ml | 100.0 ± 0.0 |
The results of the tests conducted in the table above are summarized. Regardless of the pathogen used, a significant loss of infectious particles was observed when comparing the pathogen counts measured in the culture suspension with those measured in front of the filter. The results show that the filter achieves a low reduction in infectious particles with high variation during the study. The use of UVC irradiation alone or in combination with the air filter resulted in a reduction of more than 99% in bacteria. In this study, the most efficient reduction was achieved for Actinobacillus pleuropneumoniae (i.e., 100%).
Results
UVC irradiation has been widely accepted as a potential alternative technology compared to low-pressure mercury lamps and has recently been increasingly researched. The basic characteristics and bactericidal effects were determined according to the irradiation dosages, and the inactivation mechanisms have been attempted to be clarified.
Studies conducted at the environmental scale have shown a reduction efficiency ranging from 90% to 99.999%, depending on the bacteria used for testing with UVC. However, air filters minimize the risk of airborne transmission of pathogens and can be used to reduce the pathogen load from indoor air by circulating air filtration. Moreover, since airborne dust can cause serious health problems in animals and humans and act as a carrier for pathogens, dust control is an important consideration in environments where sterility is required.
UVC irradiation (100-280 nm) is highly mutagenic for microorganisms, and its effectiveness depends on the UVC intensity (W/m²), exposure time (s), and the dose (J/m²) formed by the wavelength (254 nm). Through the formation of thymine dimers, UVC functions as a mutagen and causes damage to microbial DNA. Various studies have demonstrated the bactericidal (eliminating bacteria from the environment) effectiveness of UVC radiation. Due to the Minamata Convention, it has become mandatory to search for new technologies to replace traditional low-pressure mercury UV lamps. In this context, it is now possible to fight bacteria using UVC irradiation systems.
References
- Song K, Mohseni M, Taghipour F. 2016. Application of ultraviolet lightemitting diodes (UV-LEDs) for water disinfection: a review. Water Res 94:341–349. https://doi.org/10.1016/j.watres.2016.03.003.
- Shin J-Y, Kim S-J, Kim D-K, Kang D-H. 2016. Fundamental characteristics of deep-UV light-emitting diodes and their application to control foodborne pathogens. Appl Environ Microbiol 82:2–10. https://doi.org/10.1128/AEM.01186-15.
- Kim S-J, Kim D-K, Kang D-H. 2016. Using UVC light-emitting diodes at wavelengths of 266 to 279 nanometers to inactivate foodborne pathogens and pasteurize sliced cheese. Appl Environ Microbiol 82:11–17. https://doi.org/10.1128/AEM.02092-15.
- Kim D-K, Kim S-J, Kang D-H. 2017. Inactivation modeling of human enteric virus surrogates, MS2, Q_, and _X174, in water using UVC-LEDs, a novel disinfecting system. Food Res Int 91:115–123. https://doi.org/10.1016/j.foodres.2016.11.042.
- Ferroni A, Werkhauser-Bertrand A, Le Bourgeois M, Beauvais R, Vrielynck S, Durand C, Lenoir G, Berche P, Sermet-Gaudelus I. 2008. Bacterial contamination in the environment of hospitalised children with cystic fibrosis. J Cyst Fibros 7:477– 482. https://doi.org/10.1016/j.jcf.2008.05.001.
- Spencker F, Haupt S, Claros M, Walter S, Lietz T, Schille R, Rodloff A. 2000. Epidemiologic characterization of Pseudomonas aeruginosa in patients with cystic fibrosis. Clin Microbiol Infect 6:600–607. https://doi.org/10.1046/j.1469-0691.2000.00171.x.
- Alberti C, Bouakline A, Ribaud P, Lacroix C, Rousselot P, Leblanc T, Derouin F. 2001. Relationship between environmental fungal contamination and the incidence of invasive aspergillosis in haematology patients. J Hosp Infect 48:198 –206. https://doi.org/10.1053/jhin.2001.0998.
- Faure O, Fricker-Hidalgo H, Lebeau B, Mallaret M, Ambroise-Thomas P, Grillot R. 2002. Eight-year surveillance of environmental fungal contamination in hospital operating rooms and haematological units. J Hosp Infect 50:155–160. https://doi.org/10.1053/jhin.2001.1148.
- Perdelli F, Cristina M, Sartini M, Spagnolo A, Dallera M, Ottria G, Lombardi R, Grimaldi M, Orlando P. 2006. Fungal contamination in hospital environments. Infect Control Hosp Epidemiol 27:44–47.
- Górny RL, Dutkiewicz J. 2002. Bacterial and fungal aerosols in indoor environment in Central and Eastern European countries. Ann Agric Environ Med 9:17–23.
- Sautour M, Sixt N, Dalle F, L’Ollivier C, Fourquenet V, Calinon C, Paul K, Valvin S, Maurel A, Aho S. 2009. Profiles and seasonal distribution of airborne fungi in indoor and outdoor environments at a French hospital. Sci Total Environ 407:3766–3771. https://doi.org/10.1016/j.scitotenv.2009.02.024.
- Topbas¸ M, Tosun I, Çan G, Kaklikkaya N, Aydin F. 2006. Identification and seasonal distribution of airborne fungi in urban outdoor air in an eastern Black Sea Turkish town. Turk J Med Sci 36:31–36.
- de Ana SG, Torres-Rodríguez J, Ramírez E, García S, Belmonte-Soler J. 2006. Seasonal distribution of Alternaria, Aspergillus, Cladosporium and Penicillium species isolated in homes of fungal allergic patients. J Investig Allergol Clin Immunol 16:357–363.
- Xu Z, Wu Y, Shen F, Chen Q, Tan M, Yao M. 2011. Bioaerosol science, technology, and engineering: past, present, and future. Aerosol Sci Technol 45:1337–1349. https://doi.org/10.1080/02786826.2011.593591.
- Kim J, Jang J. 2018. Inactivation of airborne viruses using vacuum ultraviolet photocatalysis for a flow-through indoor air purifier with short irradiation time. Aerosol Sci Technol 52:557–566. https://doi.org/10.1080/02786826.2018.1431386.
- Ko G, First MW, Burge HA. 2002. The characterization of upper-room ultraviolet germicidal irradiation in inactivating airborne microorganisms. Environ Health Perspect 110:95. https://doi.org/10.1289/ehp.02110s195.
- Kujundzic E, Hernandez M, Miller SL. 2007. Ultraviolet germicidal irradiation inactivation of airborne fungal spores and bacteria in upper-room air and HVAC in-duct configurations. J Environ Eng Sci 6:1–9. https://doi.org/10.1139/s06-039.
- Walker CM, Ko G. 2007. Effect of ultraviolet germicidal irradiation on viral aerosols. Environ Sci Technol 41:5460 –5465. https://doi.org/10.1021/es070056u.
- Centers for Disease Control and Prevention. 2005. Guidelines for preventing the transmission of Mycobacterium tuberculosis in health-care settings, 2005. MMWR Recommend Rep 54(RR17):1–141.
- Bintsis T, Litopoulou-Tzanetaki E, Robinson RK. 2000. Existing and potentialapplications of ultraviolet light in the food industry—a critical review. J Sci Food Agric 80:637– 645. https://doi.org/10.1002/(SICI)1097-0010(20000501)80:6_637::AID-JSFA603_3.0.CO;2-1.
- Yaun BR, Sumner SS, Eifert JD, Marcy JE. 2004. Inhibition of pathogens on fresh produce by ultraviolet energy. Int J Food Microbiol 90:1– 8.https://doi.org/10.1016/S0168-1605(03)00158-2.
- Franz CM, Specht I, Cho G-S, Graef V, Stahl MR. 2009. UV-C-inactivation of microorganisms in naturally cloudy apple juice using novel inactivation equipment based on Dean vortex technology. Food Control 20:1103–1107. https://doi.org/10.1016/j.foodcont.2009.02.010.
- Guerrero-Beltrán J, Barbosa-Cánovas G. 2004. Advantages and limitations on processing foods by UV light. Food Sci Technol Int 10:137–147.https://doi.org/10.1177/1082013204044359.
- Lin C-Y, Li C-S. 2002. Control effectiveness of ultraviolet germicidal irradiation on bioaerosols. Aerosol Sci Technol 36:474–478. https://doi.org/10.1080/027868202753571296.
- Chang CW, Li SY, Huang SH, Huang CK, Chen YY, Chen CC. 2013. Effects of ultraviolet germicidal irradiation and swirling motion on airborne Staphylococcus aureus, Pseudomonas aeruginosa, and Legionella pneumophila under various relative humidities. Indoor Air 23:74–84. https://doi.org/10.1111/j.1600-0668.2012.00793.x.
- Seedorf J. Emissions of airborne dust and microorganisms. Landtechnik 2000; 2: 182–183.
- Holtkamp DJ, Kliebenstein JB, Neumann EJ, Zimmerman JJ, Rotto HF, Yoder TK, et al. Assessment of the economic impact of porcine reproductive and respiratory syndrome virus on United States pork producers.J Swine Health Prod. 2013; 21(2): 72–84.