Microbial Drug Resistance
Alexander Fleming discovered the first antibiotic, “penicillin,” in 1928, and when mass production became possible, it was widely used by the Allied Forces during World War II to treat wounds and postoperative infections (1). After the war, penicillin began to be prescribed to the general population, where it was widely regarded as a “miracle drug.” Scientists envisioned a future free from the threat of infectious diseases caused by bacteria. However, when Fleming won the Nobel Prize in 1945 for his discovery, he had already warned in his Nobel lecture that bacteria could “easily become resistant to penicillin” (2). This resistance had already been observed even before antibiotics were widely used in clinical practice. Since then, many antibiotics have been discovered, and thanks to them, terrible infectious diseases caused by bacteria (such as pneumonia, tuberculosis, cholera, typhoid, and diphtheria) did not lead to pandemics like in ancient times. Antibiotics made surgery, trauma from accidents, and childbirth much safer.
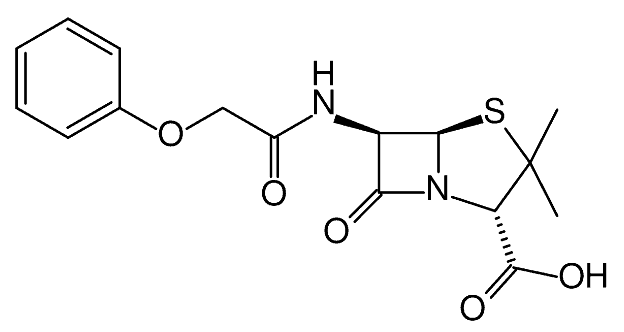
However, in the last 60 years, the development of antibiotic resistance has accelerated rather than slowed down (Table 1). The reasons for this are numerous and varied. Global antibiotic consumption in clinical medicine increased by about 40% between 2000 and 2010. However, this figure masks declining usage patterns in some countries and rapid growth in others. There are significant differences between countries. The annual per capita antibiotic consumption varies more than 10-fold, even in developed countries. The excessive and inappropriate use of antibiotics is facilitated in many places due to their availability without a prescription, and in some regions, the market contains fake and substandard antibiotics. The speed and volume of international travel create new opportunities for the global spread of antibiotic-resistant pathogens. Bacteria can easily share genetic material with one another and create new resistant strains at an unprecedented rate.
As noted by Gloria Guglielmi, nearly 80% of all antibiotics in the United States are not used by humans, but in livestock feed, which is the biggest problem (3). Cows, pigs, and chickens are given antibiotics to promote faster growth and to keep the animals healthy under naturally unhealthy factory farm conditions. In 2013, more than 131,000 tons of antibiotics were used in food animals worldwide, and this is expected to exceed 200,000 tons by 2030.
Antibiotic introduced | Antibiotic resistance identified |
1943 penisilin | 1943 penicillin-resistant Staphylococcus |
1950 tetracycline | 1959 tetracycline-resistant Shigella |
1953 erythromycin | 1978 erythromycin-resistant Pneumococcus |
1960 Meticillin | 1962 methicillin-resistant Staphylococcus |
1967 Gentamicin | 1979 Gentamicin-resistant Enterococcus |
1972 Vancomycin | 1988 Vancomycin-resistant Enterococcus |
1985 Cefotaxime | 1987 Cefotaxime-resistant Enterobacteriaceae |
1985 Imipenem | 1998 Imipenem-resistant Enterobacteriaceae |
1996 Lefloxacin | 1996 Lefloxacin-resistant Pneumococcus |
2000 linezolid | 2001 linezolide dirençli Staphylococcus |
2003 daptomisin | 2005 daptomisine dirençli Staphylococcus |
2010 seftarolin | 2011 seftaroline dirençli Staphylococcus |
Table 1. Timeline of New Antibiotics Release and Emergence of First Resistance Reports
The true value of antibiotics goes beyond preventing infection-related death and disease; antibiotics allow for serious iatrogenic assaults in cancer treatments like chemotherapy or radiation therapy, or in organ transplants, by keeping the complication rates from immune system issues low (4). Therefore, antibiotics are an extremely valuable resource in the entire spectrum of modern medicine (5). However, multi-drug-resistant and pan-drug-resistant bacterial strains and the infections they cause have become emerging threats to global public health (6). These infections are associated with nearly double the mortality rate and significantly longer hospital stays (7). The treatment of infections caused by antibiotic-resistant microbes is often exceptionally difficult due to the limited number of treatment options (8). Therefore, there is an urgent need for comprehensive research into alternative antimicrobial approaches to kill multi-drug-resistant strains, focusing on methods that are less likely to cause resistance development (9-11). Recently, Karen Bush and colleagues pointed out that new non-antibiotic approaches to prevent and treat infectious diseases should be considered high-priority international research and development goals (12).
To achieve this goal, a promising, innovative approach is the use of light-based methods to inactivate pathogenic and resistant microbes infecting living tissues, without causing unacceptable damage to those tissues.
Safe and Effective UVC
One of the attractive features of UV technology is the ability to select a preferred wavelength that can be effectively applied to control a target pathogen or other specific applications, as various monochromatic wavelengths can be produced depending on the UV source. Among the typical UV sources that can produce various wavelengths, the UVC 254 nm low-pressure mercury lamps are the most preferred. Due to the different mechanisms involved, combining wavelengths that have a synergistic effect on microbial inactivation can be applied with innovative technology (13). As a result, with the combination representing the synergistic effect, it is possible to reduce the input energy by lowering the required intensity to reduce the pathogen to the desired level, ensuring safety against pathogens with an increased inactivation effect (14, 15).
Many studies have been conducted on the synergistic effects of different wavelengths for microbial inactivation. Among them, the most preferred are the inactivation effects of UVC 254 nm wavelength low-pressure mercury lamps (16, 17).
UVC light is electromagnetic radiation in the range of 200 to 280 nm wavelengths. UV light, especially UVC, has long been known for its high antiseptic properties (18). UVC light with a 254 nm wavelength is easily produced from a low-pressure mercury vapor lamp and is commonly used to inactivate and kill various microbial species, often referred to as germicidal UV (19). The antimicrobial mechanism of UVC light relies on its ability to damage DNA by inducing various mutagenic and cytotoxic DNA lesions, such as cyclobutane pyrimidine dimers and 6,4 photo-products (20, 21). As shown in Figure 2, the transcription, translation, and replication of DNA are affected by this process, leading to cell death (22). It has been reported that irradiating surgical areas with antiseptic UVC during orthopedic surgical procedures reduces the rate of surgical site infections (23).
Recent studies with 254 nm light-emitting antiseptic UVC lamps are promising for combating biological hazards in the environment, infection control, and achieving sterilized environments (24).
UVC against Antibiotic Resistance in Bacteria
Gram-positive bacteria are generally more easily inactivated by UVC light than gram-negative bacteria. This difference in susceptibility is linked to the distinct compositions of their cell walls. Gram-positive bacteria have a relatively porous cell wall made up of multiple layers of peptidoglycan, allowing molecules with molecular weights of 1500 to 1800 Da to pass through easily. In contrast, gram-negative bacteria possess an additional, highly organized outer membrane outside the peptidoglycan layer. The asymmetric nature of this outer membrane, composed of phospholipids, proteins, lipoproteins, and negatively charged lipopolysaccharides, prevents the passage of various molecules through it, including those of smaller molecular weights (600-700 Da).
The underlying principle of “safe UVC” is based on the differential absorption of UVC photons by proteins and nucleic acids. While proteins have a logarithmic absorption coefficient at 254 nm, nucleic acids exhibit a much higher absorption coefficient. As a result, UVC light at this wavelength has a higher probability of damaging the DNA molecule, leading to inactivation. Since prokaryotic bacteria lack a membrane-bound nucleus, their DNA is exposed and more directly accessible to UVC light, making them highly sensitive to DNA damage.
Recent studies have shown that bacteria commonly found in hospital environments, such as S. aureus, MRSA, P. aeruginosa, Klebsiella pneumoniae, Clostridium difficile, Streptococcus pyogenes, Mycobacterium spp., and Salmonella, possess endogenous photosensitizing chromophores that make them susceptible to UVC light at 254 nm. Notably, gram-positive bacteria are considered more sensitive to UVC light than gram-negative bacteria.
One of the major drawbacks of antibiotics is the emergence of resistance over time, which leads to the inability to treat infections caused by multi-drug resistant organisms. In contrast, UVC light, especially at 254 nm, is effective against a wide variety of pathogens, regardless of their resistance to conventional antibiotics. Research has indicated that the likelihood of bacteria developing resistance to UVC light is very low. For instance, studies by Guffey found that S. aureus is unlikely to develop resistance to light therapy if used correctly. Other studies have also shown that resistance to 254 nm UVC is not likely to develop (35,36).
Multiple studies have confirmed the effectiveness of 254 nm UVC in inactivating pathogenic and antibiotic-resistant bacteria. Recent work by Huang et al. showed a reduction of 4-5 log10 in E. coli colony-forming units (CFU) after UVC treatment (37). Similarly, Fila et al. successfully reduced wild-type and multi-drug-resistant P. aeruginosa by up to 8 log10 CFU (38).
In a study by Wang et al., the antimicrobial activity of 254 nm UVC against gram-negative pathogens in biofilms was confirmed, with reductions of 5.59 log10 CFU A. baumannii biofilm and 5.02 log10 CFU P. aeruginosa (31). Halstead et al. showed that 34 different planktonic-phase bacteria, including K. pneumoniae and E. faecium, were sensitive to 254 nm UVC, with 71% of them experiencing a 5 log10 CFU reduction after 15-30 minutes of exposure. Biofilms also experienced significant reductions (39).
Despite the higher light dose required for spore inactivation, Moorhead et al. demonstrated the successful inactivation of C. difficile vegetative cells and spores, reducing them by 6 log10 CFU (40). Zhang et al. reported that a single exposure to UVC light led to the inactivation of 6.75 log10 CFU of C. albicans (41). Wang et al. (2017) conducted a review confirming that over 47 different pathogens commonly associated with hospital-acquired infections were successfully inactivated by 254 nm UVC light (42).
In conclusion, UVC light, especially at the 254 nm wavelength, offers a promising solution for combating antibiotic-resistant bacteria. Its ability to effectively inactivate a broad range of pathogens, regardless of their resistance profiles, positions it as a powerful tool in infection control and sterilization, particularly in healthcare settings. However, further research and controlled application are essential to optimize its use and ensure safety.
Results
One of the fundamental advantages of light-based approaches, such as UVC, is their broad-spectrum nature. These methods are effective in killing or neutralizing a wide range of microorganisms, including bacteria (both gram-positive and gram-negative), mycobacteria, fungi (yeasts and molds), viruses (DNA and RNA types), and parasites.
This study has discussed various UVC applications that can be used in the fight against antibiotic-resistant bacteria. Recent research has emphasized the versatility of light-based technologies in addressing pathogens across all known microbial classes. UVC light can be used to kill or inactivate gram-positive and gram-negative bacteria, fungi, endospores, parasites, viruses, and even protein toxins. The mechanisms of action depend on the microbial type, the wavelength of UVC light, and its presence. The wide applicability of light-based technologies to biological warfare agents suggests that such technologies are likely very broad-spectrum, eliminating the need to identify specific agents in the event of a mass biological attack. Moreover, resistance to light-based inactivation is not likely to develop, even in antibiotic-resistant bacteria.
An additional benefit of UVC light is that it is non-polluting and environmentally friendly. The use of light-based technologies for environmental sterilization suggests that they could be useful for decontaminating areas exposed to biological agents without creating environmental pollution. This makes light-based inactivation not only effective but also sustainable. Furthermore, because light-based inactivation targets only harmful biological entities (such as pathogens), it does not affect cells that contain antigens, providing a more selective approach to disinfection.
UVC light’s significant advantage is that it has been proven to prevent the artificial development of antibiotic-resistant bacteria. Repeated application of sub-lethal doses of UVC light does not lead to the creation of resistant bacteria, as the bacteria cannot regrow in response to this treatment. This makes UVC a promising alternative to traditional antibiotics, especially in the face of the growing issue of antimicrobial resistance.
In conclusion, light-based technologies like UVC offer a broad spectrum of effectiveness against various pathogens, including antibiotic-resistant bacteria, and present an environmentally friendly solution for disinfection. Given the inability of pathogens to develop resistance to UVC light, it stands as a crucial tool in the fight against infections, including those caused by multi-drug resistant organisms.
REFERENCES
- Fleming A (1929). On the Antibacterial Action of Cultures of a Penicillium, with Special Reference to their Use in the Isolation of B. influenzæ. Br J Exp Pathol 10, 226–236.
- Fleming A (12 11, 1945). Penicillin. Nobel Lecture.
- Guglielmi G (2017). Are antibiotics turning livestock into superbug factories? Science.
- Youngblood WJ, Gryko DT, Lammi RK, Bocian DF, Holten D and Lindsey JS. (2002). Glaser-mediated synthesis and photophysical characterization of diphenylbutadiyne-linked porphyrin dyads. J Org Chem. 67, 2111–2117.
- Smith R and Coast J. (2013). The true cost of antimicrobial resistance. Br Med J. 346, f1493.
- Kraus CN (2008). Low hanging fruit in infectious disease drug development. Curr Opin Microbiol. 11, 434–438.
- Munoz-Price LS, Poirel L, Bonomo RA, Schwaber MJ, Daikos GL, Cormican M, Cornaglia G, Garau J, Gniadkowski M, Hayden MK, Kumarasamy K, Livermore DM, Maya JJ, Nordmann P, Patel JB, Paterson DL, Pitout J, Villegas MV, Wang H, Woodford N and Quinn JP. (2013). Clinical epidemiology of the global expansion of Klebsiella pneumoniae carbapenemases. Lancet Infect Dis. 13, 785–796.
- Yoneyama H and Katsumata R. (2006). Antibiotic resistance in bacteria and its future for novel antibiotic development. Biosci Biotechnol Biochem. 70, 1060–1075.
- Hamblin MR and Hasan T. (2004). Photodynamic therapy: a new antimicrobial approach to infectious disease? Photochem Photobiol Sci. 3, 436–450.
- Maisch T (2007). Anti-microbial photodynamic therapy: useful in the future? Lasers Med Sci. 22, 83–91.
- Maisch T, Hackbarth S, Regensburger J, Felgentrager A, Baumler W, Landthaler M and Roder B. (2011). Photodynamic inactivation of multi-resistant bacteria (PIB) – a new approach to treat superficial infections in the 21st century. J Dtsch Dermatol Ges. 9, 360–366.
- Bush K, Courvalin P, Dantas G, Davies J, Eisenstein B, Huovinen P, Jacoby GA, Kishony R, Kreiswirth BN, Kutter E, Lerner SA, Levy S, Lewis K, Lomovskaya O, Miller JH, Mobashery S, Piddock LJ, Projan S, Thomas CM, Tomasz A, Tulkens PM, Walsh TR, Watson JD, Witkowski J, Witte W, Wright G, Yeh P and Zgurskaya HI. (2011). Tackling antibiotic resistance. Nat Rev Microbiol. 9, 894–896.
- Rahman S, Khan I, Oh DH. 2016. Electrolyzed water as a novel sanitizer in the food industry: current trends and future perspectives. Comp Rev Food Sci Food Safety 15:471– 490. https://doi.org/10.1111/1541-4337.12200.
- Leistner L. 2000. Basic aspects of food preservation by hurdle technology. Int J Food Microbiol 55:181–186. https://doi.org/10.1016/S0168-1605(00)00161-6.
- Khan I, Tango CN, Miskeen S, Lee BH, Oh D-H. 2017. Hurdle technology: a novel approach for enhanced food quality and safety—a review. Food Control 73:1426 –1444. https://doi.org/10.1016/j.foodcont.2016.11.010.
- Ramsay IA, Niedziela J-C, Ogden ID. 2000. The synergistic effect of excimer and low-pressure mercury lamps on the disinfection of flowing water. J Food Prot 63:1529 –1533. https://doi.org/10.4315/0362-028X-63.11.1529.
- Chevremont A-C, Farnet A-M, Coulomb B, Boudenne J-L. 2012. Effect of coupled UV-A and UV-C LEDs on both microbiological and chemical pollution of urban wastewaters. Sci Total Environ 426:304 –310. https://doi.org/10.1016/j.scitotenv.2012.03.043.
- Gupta A, Dai T, Avci P, Huang YY and Hamblin MR. (2013). Ultraviolet Radiation in Wound Care: Sterilization and Stimulation Adv Wound Care. doi:10.1089/wound.2012.0366.,doi:
- Chang JC, Ossoff SF, Lobe DC, Dorfman MH, Dumais CM, Qualls RG and Johnson JD. (1985). UV inactivation of pathogenic and indicator microorganisms. Appl Environ Microbiol. 49, 1361–1365.
- Poepping C, Beck SE, Wright H and Linden KG. (2014). Evaluation of DNA damage reversal during medium-pressure UV disinfection. Water Res. 56, 181–189.
- McCready S (2014). An immunoassay for measuring repair of UV photoproducts. Methods Mol Biol. 1105, 551–564.
- Gurzadyan GG, Gorner H and Schulte-Frohlinde D. (1995). Ultraviolet (193, 216 and 254 nm) photoinactivation of E. coli strains with different repair deficiencies. Radiat Res. 141, 244–251.
- Ritter MA, Olberding EM and Malinzak RA. (2007). Ultraviolet lighting during orthopaedic surgery and the rate of infection. J Bone Joint Surg Am. 89, 1935–1940.
- Dai T, Garcia B, Murray CK, Vrahas MS and Hamblin MR. (2012). UVC Light Prophylaxis for Cutaneous Wound Infections in Mice. Antimicrob Agents Chemother. 56, 3841–3848.
- Jori, G.; Fabris, C.; Soncin, M.; Ferro, S.; Coppellotti, O.; Dei, D.; Fantetti, L.; Chiti, G.; Roncucci, G. Photodynamic therapy in the treatment of microbial infections: Basic principles and perspective applications. Lasers Surg. Med. 2006, 38, 468–481.
- Maisch, T.; Szeimies, R.M.; Jori, G.; Abels, C. Antibacterial photodynamic therapy in dermatology. Photochem. Photobiol. Sci. 2004, 3, 907–917.
- Nikaido, H. Prevention of drug access to bacterial targets: Permeability barriers and active e_ux. Science 1994, 264, 382–388.
- Dai T, Kharkwal GB, Zhao J, St Denis TG, Wu Q, Xia Y, Huang L, Sharma SK, d’Enfert C and Hamblin MR. (2011). Ultraviolet-C light for treatment of Candida albicans burn infection in mice. Photochem Photobiol. 87, 342–349. [PubMed: 21208209]
- Eliasson B and Kogelschatz U. (1988). UV excimer radiation from dielectric-barrier discharges, Appl. Phys. B Lasers Opt. 46, 299–303.
- Maclean, M.; McKenzie, K.; Anderson, J.G.; Gettinby, G.; MacGregor, S.J. 405 nm light technology for the inactivation of pathogens and its potential role for environmental disinfection and infection control. J. Hosp. Infect. 2014, 88, 1–11.
- Wang, Y.;Wu, X.; Chen, J.; Amin, R.; Lu, M.; Bhayana, B.; Zhao, J.; Murray, C.K.; Hamblin, M.R.; Hooper, D.C.; et al. Antimicrobial Blue Light Inactivation of Gram-Negative Pathogens in Biofilms: In Vitro and In Vivo Studies. J. Infect. Dis. 2016, 213, 1380–1387.
- Zhang, Y.; Zhu, Y.; Gupta, A.; Huang, Y.; Murray, C.K.; Vrahas, M.S.; Sherwood, M.E.; Baer, D.G.; Hamblin, M.R.; Dai, T. Antimicrobial blue light therapy for multidrug-resistant Acinetobacter baumannii infection in a mouse burn model: Implications for prophylaxis and treatment of combat-related wound infections. J. Infect. Dis. 2014, 209, 1963–1971.
- Dai, T.; Gupta, A.; Huang, Y.Y.; Sherwood, M.E.; Murray, C.K.; Vrahas, M.S.; Kielian, T.; Hamblin, M.R. Blue light eliminates community-acquired methicillin-resistant Staphylococcus aureus in infected mouse skin abrasions. Photomed. Laser Surg. 2013, 31, 531–538.
- Gu_ey, J.S.; Payne, W.; Martin, K.; Dodson, C. Delaying the onset of resistance formation: E_ect of manipulating dose, wavelength, and rate of energy delivery of 405-, 464-, and 850-nanometer light for Staphylococcus aureus. Wounds 2014, 26, 95–100.
- Tomb, R.M.; Maclean, M.; Coia, J.E.; MacGregor, S.J.; Anderson, J.G. Assessment of the potential for resistance to antimicrobial violet-blue light in Staphylococcus aureus. Antimicrob. Resist. Infect. Control 2017, 6, 100–112.
- Yin, R.; Dai, T.; Avci, P.; Jorge, A.E.; de Melo, W.C.; Vecchio, D.; Huang, Y.Y.; Gupta, A.; Hamblin, M.R. Light based anti-infectives: Ultraviolet C irradiation, photodynamic therapy, blue light, and beyond. Curr. Opin. Pharmacol. 2013, 13, 731–762.
- Huang, S.T.; Wu, C.Y.; Lee, N.Y.; Cheng, C.W.; Yang, M.J.; Hung, Y.A.; Wong, T.W.; Liang, J.Y. E_ects of 462 nm Light-Emitting Diode on the Inactivation of Escherichia coli and a Multidrug-Resistant by Tetracycline Photoreaction. J. Clin. Med. 2018, 7, 1–15.
- Fila, G.; Kawiak, A.; Grinholc, M.S. Blue light treatment of Pseudomonas aeruginosa: Strong bactericidal activity, synergism with antibiotics and inactivation of virulence factors. Virulence 2017, 8, 938–958.
- Halstead, F.D.; Thwaite, J.E.; Burt, R.; Laws, T.R.; Raguse, M.; Moeller, R.;Webber, M.A.; Oppenheim, B.A. Antibacterial Activity of Blue Light against Nosocomial Wound Pathogens Growing Planktonically and as Mature Biofilms. Appl. Environ. Microbiol. 2016, 82, 4006–4016.
- Moorhead, S.; MacLean, M.; Coia, J.; MacGregor, S. Inactivation of C. di_cile by 405 nm HINS-light. In Proceedings of the 8th Annual Scottish Environmental and Clean Technology Conference, Glasgow, Scotland, 26 June 2014.
- Zhang, Y.; Zhu, Y.; Chen, J.; Wang, Y.; Sherwood, M.E.; Murray, C.K.; Vrahas, M.S.; Hooper, D.C.; Hamblin, M.R.; Dai, T. Antimicrobial blue light inactivation of Candida albicans: In vitro and in vivo studies. Virulence 2016, 7, 536–545.
- Wang, Y.;Wang, Y.;Wang, Y.; Murray, C.K.; Hamblin, M.R.; Hooper, D.C.; Dai, T. Antimicrobial blue light inactivation of pathogenic microbes: State of the art. Drug Resist. Updat. 2017, 33–35, 1–22.